The emission of ozone-depleting gases impacted the earth’s ozone layer – a layer that absorbs most of the Sun’s ultraviolet radiation. Emissions were increasing rapidly until the 1980s.
Since then the world has achieved rapid progress: the near-elimination of ozone-depleting substances and the trend towards recovery of the ozone layer are arguably among the most successful international environmental collaborations to date. In this entry we present the data on ozone layer depletion, signs of recovery, emissions of ozone-depleting substances, international agreement and collaboration, and the consequences of ozone layer depletion.
Summary
- Global emissions of ozone-depleting substances have declined by more than 99% since 1986 (the year before international action was agreed).
- The Montreal Protocol (and later amendments) was adopted in 1987 — since then all countries have signed on to the agreement, allowing for the dramatic decline in global ozone-depleting emissions.
- Ozone layer thickness declined, and the Antarctic ozone hole grew substantially from the 1980s through to the early 2000s. Through the first decade this trend largely stabilized and we now see initial signs of recovery.
- It will take decades for the ozone layer to recover.
- Ozone layer depletion increases the amount of ultraviolet (UV) irradiation that reaches Earth’s surface; this can increase the risk of skin cancer, particularly at higher latitudes.
- The global shift away from ozone-depleting substances has also had co-benefits on the reduction of greenhouse gas emissions.
All our charts on Ozone Layer
- Annual change in atmospheric CFC-11 concentrations
- Antarctic ozone hole area
- Change in the consumption of ozone-depleting substances
- Consumption of Ozone-Depleting Substances
- Countries subscribed to the Montreal Protocol
- Equivalent stratospheric chorine (ESC) concentrations
- Excess skin cancer cases due to ozone depletion
- Measured and expected atmospheric concentrations of CFC-11
- Number of parties in multilateral environmental agreements
- Ozone-depleting substance consumption
- Ozone-depleting substance emissions
- Stratospheric ozone concentration
- Stratospheric ozone concentration projections
- Subscriptions to the Montreal Protocol by region
Why is the ozone layer important?
What is ozone?
Ozone (O3) is a gas that is present naturally within Earth’s atmosphere. It is formed of three oxygen atoms (giving it the chemical formula, O3). Its structure means that it’s much less stable than oxygen (O2), and is therefore much more reactive; this means it can be more easily formed and broken down through interaction with other compounds.
This reactivity is significant in its interactions described in the entry below: the ozone layer can be depleted and broken down through its interaction with man-made compounds in the upper atmosphere.
Ozone and its impact on the atmosphere
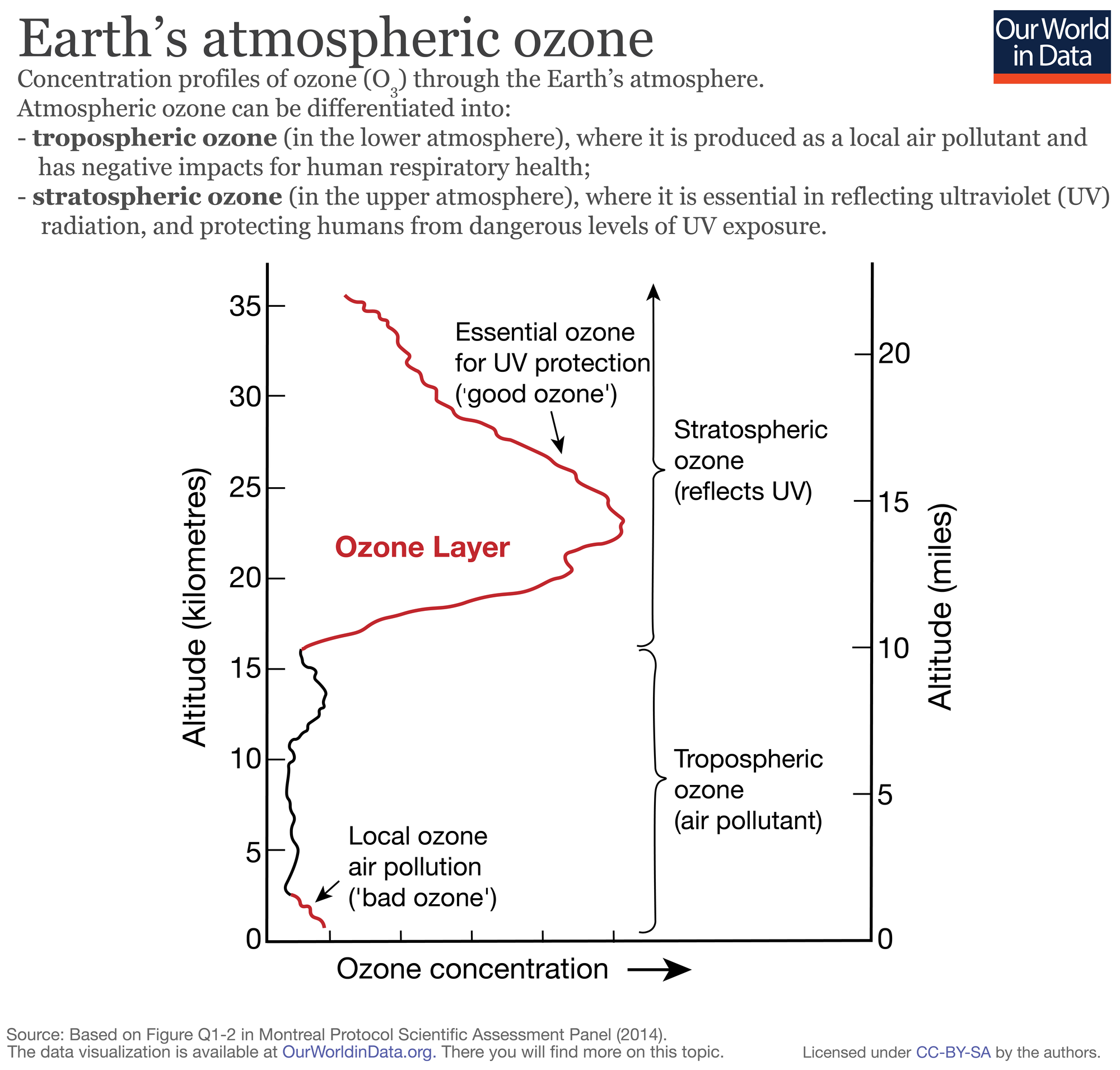
To understand the importance of the ozone layer, it’s crucial to first establish the difference in concentrations of ozone throughout the Earth’s atmosphere.
In the figure we see a standard profile of ozone gas concentration through the Earth’s atmosphere, extending from ground level up to 40 kilometres in altitude. Ozone plays a different role in atmospheric chemistry at different heights in the Earth’s atmosphere. We can differentiate this profile into two key zones:
- Tropospheric ozone is that which is present in the lower atmosphere (the troposphere; with a height of 5km to 15km depending on your latitude). Throughout most of the troposphere, ozone concentrations are relatively low (as shown in the diagram). However, concentrations of ozone can be higher very close to the surface at local levels; there it forms as an air pollutant and can negatively impact human health. Ground-level ozone can form through chemical reactions between local air pollutants such as nitrous oxides (NOx), volatile organic compounds (VOCs) and sunlight. These air pollutants are emitted from motor vehicle exhausts, industrial processes, electric utilities, and chemical solvents. Ground-level ozone can have negative impacts on human health; breathing ozone is particularly harmful to the young, elderly and people with underlying respiratory problems. It is therefore commonly referred to as ‘bad’ ozone.
- Stratospheric ozone is that which is present in the upper atmosphere (the stratosphere; typically extending from around 10 to 15km up to 50km depending on latitude). As shown in the diagram, concentrations of ozone are higher in the stratosphere than in the troposphere. The stratosphere includes the zone termed the ‘ozone layer’.Ozone in the stratosphere plays a very different role to that in the layer below. In the ozone layer, it is often referred to as ‘good’ ozone since it plays a crucial role in absorbing potentially dangerous ultraviolet (UV-B) radiation from the sun. The ozone layer typically absorbs 97-99 percent of incoming UV-B radiation. Higher concentrations of ozone in the stratosphere is therefore crucial to ensure life (including humans) at Earth’s surface are not exposed to harmful concentrations of UV-B radiation.
The focus of this entry will be on the ‘ozone layer’ — the ozone that exists within the stratosphere. The impact of ozone in the troposphere (as a local air pollutant) is instead covered in our entry on Air Pollution.
What are ozone-depleting substances?
Ozone-depleting substances (ODS) are halogen gases containing chlorine and/or bromine which have the potential to break down ozone in the stratosphere. There are a significant number of ODS, including chlorofluorocarbons (CFCs), hydrochlorofluorocarbons (HCFCs), methyl chloride and bromide, and halons.
These gases, emitted at the surface, are distributed globally through the lower atmosphere through wind transport patterns. From there, they can be transported into the upper atmosphere (stratosphere) where they can form highly reactive chlorine or bromine gases in the presence of ultraviolet (UV) sunlight. Reactive halogen gases can then destroy stratospheric ozone, resulting in depletion of the ozone layer.
Individual ozone-depleting substances are not equal in their impact on depletion. Some gases, per tonne, have a significantly higher potential for ozone depletion than others. In our section on Data Quality & Definitions, we provide a table of ODS and their respective ‘ozone depleting potential’ (ODP). ODP measures the relative impact on ozone depletion per tonne of gas. An ODP of 10, for example, is ten times as effective in depleting ozone per tonne, than an ODP of 1.
Emissions of ozone-depleting substances
Total emissions of ozone-depleting substances
Ozone-depleting substances (chlorines and bromines) can be emitted from natural and anthropogenic (man-made) sources. In the chart, we see emissions of ozone-depleting substances from 1960 onwards. This is measured in tonnes of chlorofluorocarbon-11-equivalents (CFC11 equivalents). CFC11 equivalents is a standardized measure to normalize the sum of a range of ozone-depleting substances to a value equivalent to their potential to deplete ozone; as we describe in our Data Quality & Definitions section, depleting substances are weighted by their potential to destroy ozone.
Shown in the chart is the level of natural emissions (which has been approximately consistent over this period), and total emissions which is the sum of natural and man-made emissions. Here we see a clear growth-peak-reduction trend in ozone-depleting emissions, with a rapid rise in emissions (increasing more than three-fold) from 1960 through to the late 1980s, followed by a similarly fast reduction in the decades which followed. By 2010, emissions had returned to 1960 levels. This was largely the result of international regulatory agreements and concerted action to phase-out the production and consumption of these substances (explored later in this entry).
Production/consumption of ozone-depleting substances
IN THIS SECTION
- Consumption by country
- Consumption by substance
In the section above we provide data on global emissions of ozone-depleting substances. Emissions do not necessarily equate to the production/consumption of these materials; in our section on Data Quality & Definitions, we explain the distinction between these two measures.
In the chart, we see the magnitude of a global decline in ODS consumption since 1986. This data measures the indexed consumption of ODS to 1986 (i.e. levels in 1986 are equal to 100). Following a 28% rise in 1987, we see a rapid consumption in global emissions. Consumption fell by more than 60% by 1995; 80% by 2000; and by 99.7% percent by 2018.
Consumption by country
In the chart, we see the quantity of ODS consumption by country. This is measured in tonnes of ozone-depleting substances all weighted relative to their depleting potential.
Using the ‘play’ button on the map allows you to view changes across the world since 1989. By clicking on a country on the map, you can view a time series of how its national consumption has changed over this period.
Consumption by substance
The trends in consumption above have been aggregated to the total consumption of ODS. This quantifies the aggregate of a number of substances. In the chart, we see the breakdown of consumption by substance. Note that, as with other measures throughout this entry, each substance has been weighted by its potential to destroy ozone.
By using the “change country” button in the interactive chart you can view consumption patterns of individual substances by country or region. By checking the ‘relative’ box you can also view the percentage share of a given substance to total ODS consumption.
At the global level, we see the trend of declining consumption — as established above — since 1989. However, it’s also interesting to note the relative decline and change in the number of individual substances. Throughout the 1990s and first half of the 2000s, chlorofluorocarbons (CFCs) dominated global consumption (accounting for 60 percent, reducing to 50 percent). However, through the 2000s we have seen a rising dominance of hydrochlorofluorocarbons (HCFCs); in 2014 HCFCs accounted for 94 percent of global consumption.
This transition reflects the substitution of CFCs with HCFCs in order to reduce total ozone depletion. When we compare the ozone-depleting potential (ODP) of CFCs and HCFCs we see that the depleting potential of one tonne or HCFCs is 10 to 100 times less than that of one tonne of CFCs. This replacement was therefore been an important reduction strategy (particularly where the complete phase-out of ozone-depleting substances was not readily available).
Chlorofluorocarbons (CFCs) have almost been completely phased out, declining from over 800,000 tonnes in 1989 to 156 tonnes in 2014.
International agreements
Vienna Convention for the Protection of the Ozone Layer
The rapid decline in emissions of ozone-depleting substances shown above was driven by international agreement to phase out their production. In 1985 the Vienna Convention for the Protection of the Ozone Layer was adopted and entered into force in 1988.1
In the chart we see the evolution of global parties signing on to the Vienna Convention. In its first year (1988) there were only 29 parties signed to the agreement. This rapidly increased in the years to follow, reaching 174 parties by 2000. In 2009, the Vienna Convention became the first of any Convention to achieve universal ratification.
The Vienna Convention, despite not mandating parties to take concrete actions on ozone protection laid the foundations for adoption of The Montreal Protocol.
Montreal Protocol
The Montreal Protocol on Substances that Deplete the Ozone Layer is arguably the most successful international treaty to date.
The Montreal Protocol is an international protocol to the Vienna Convention, agreed in 1987 before entering into force in 1989.
Its purpose was to phase out (reduce and eventually eliminate) the use of man-made ozone-depleting substances for the protection of the ozone layer.
Using the ‘play’ button and timeline in the chart we can observe how the Montreal Protocol was adopted across the world since 1987. The Protocol has now reached universal ratification, with South Sudan as the final signatory in 2012.
Since its first draft in 1987, the Montreal Protocol has undergone numerous amendments of increasing ambition and reduction targets. As shown in the following section, subsequent amendments were been critical in the Protocol’s success in reducing ODS consumption.
Impact of the Montreal Protocol
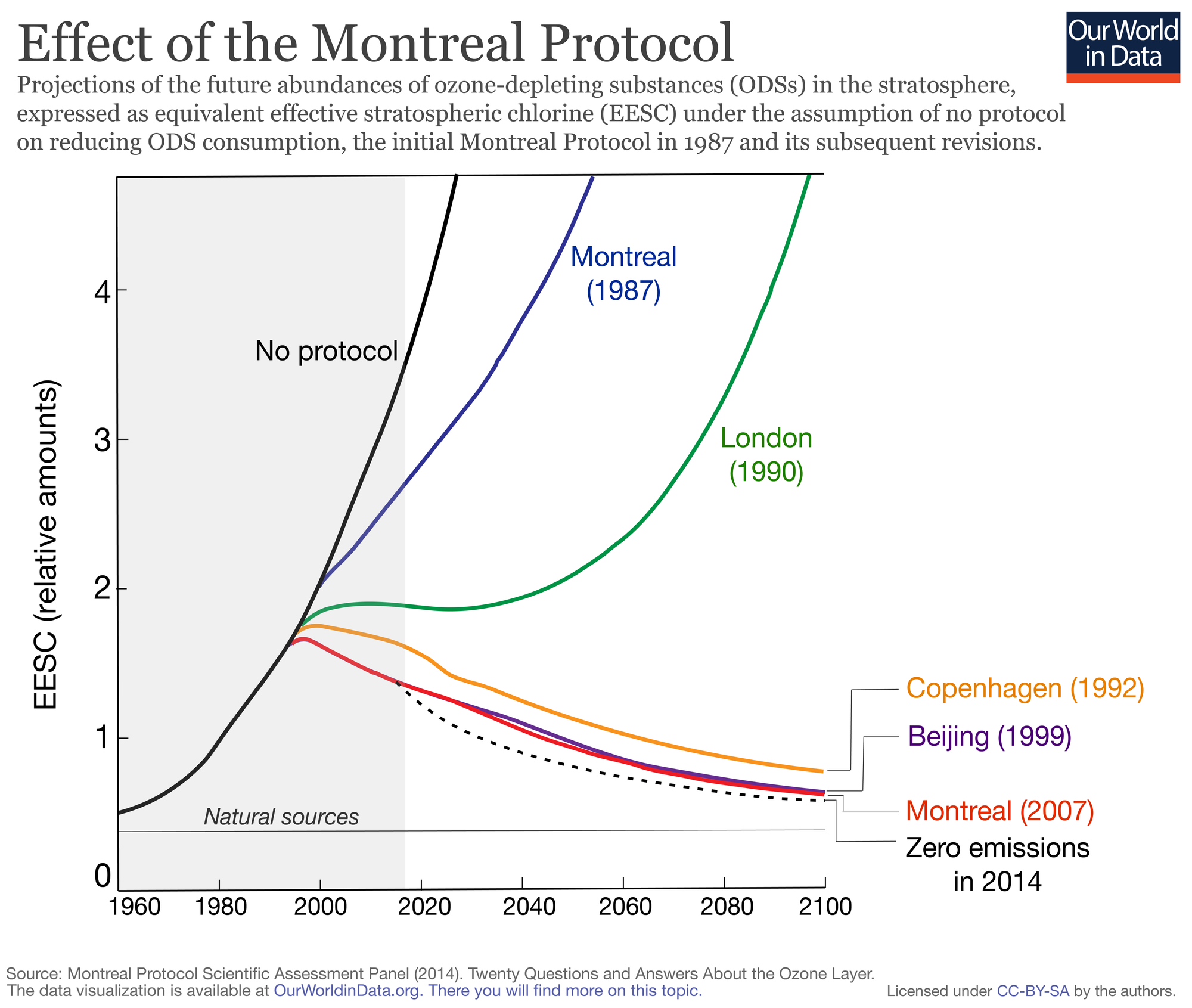
How critical was the Montreal Protocol in reducing ODS consumption and stratospheric concentrations?
In the chart, we see various projections of historic and future concentrations of effective chlorine substances (i.e. depleting substances) under evolving protocol scenarios. These are mapped from assumptions of no international protocol, the first Montreal treaty in 1987, followed by subsequent revisions of increasing ambition.
As shown, under the instance of no protocol, it’s projected that global ODS emissions and stratospheric concentrations would have continued to increase rapidly in the decades to follow. However, even under the initial Montreal Protocol, and subsequent London (1990) amendment, reduction controls and targets would have been too relaxed to have resulted in a reduction in ODS emissions. A reduction or slowdown in emissions relative to a ‘no protocol’ scenario would have been achieved but this would be insufficient to lead to an absolute reduction.
However, Copenhagen (1992) and its subsequent revisions greatly increased controls and ambition in global commitments, leading to a peak in stratospheric concentrations in the early 2000s and projected declines in the decades to follow.
Ozone layer depletion
IN THIS SECTION
- Stratospheric ozone concentration
- Ozone hole area
Stratospheric ozone concentration
What impact have man-made ODS emissions had on stratospheric ozone concentrations?
In the chart, we see average stratospheric ozone concentrations in the Southern Hemisphere (where ozone depletion has been most severe) from 1979 to 2017. Ozone concentrations are collected via satellite instruments as part of NASA’s Ozone Watch meteorological programme.
Ozone concentrations are measured in Dobson Units (DU): this is the number of molecules of ozone that would be required to create a layer of pure ozone 0.01 millimetres thick at a temperature of 0 degrees Celsius and a pressure of 1 atmosphere. An ‘Ozone Hole’ would approximate an area where the ozone concentration drops to an average of around 100 Dobson Units.
We see that from 1979 through to the early 1990s, stratospheric ozone concentrations in the South Hemisphere fell to the concerning ‘ozone hole’ level of 100 DU. For several decades since the 1990s, concentrations have continued to approximate around (or below) 100 DU. Over the last few years since 2010, however, ozone concentrations have started to slowly recover.
Ozone hole area
Has the fall of stratospheric ozone concentrations been reflected in an ozone hole? In the chart, we see the maximum and mean ozone hole area over Antarctica, measured in square kilometres (km2). Like gas concentrations, the ozone hole area is monitored daily by NASA via satellite instruments.
Since 1979 we see a distinct increase in the Antarctic ozone hole area, reaching a maximum of 30 million km2 in the early 2000s. However, since the late 1990s, the ozone hole area had approximately stabilized between 20 to 25 million km2. In 2018, the NASA Aura Program published its first results to shown clear initial signs of ozone hole recovery.4
Full recovery is, however, expected to take until (at least) the second half is this century (as described in the entry below).
Satellite and data imaging of the Antarctic ozone hole from 1979 through to 2017 can be viewed at NASA’s Goddard Media Centre; this provides a very visual understanding of the growth of the Antarctic ozone hole over this period.
When is the ozone layer expected to recover?
The Ozone Layer has recently shown early signs of recovery.5
However, full recovery of stratospheric ozone concentrations to historical levels is projected to take many more decades.
In the charts, we profile historic levels and future projections of recovery in two forms: equivalent stratospheric chlorine (i.e. ODS) concentrations, and stratospheric ozone concentrations through to 2100. This is measured as the global average, as well as concentrations in Antarctic and Arctic zones. Note that such projections are given as the median lines from a range of chemistry-climate; true modelled results presented in the Montreal Protocol Scientific Assessment Panel (2014) report present the full range of modelled estimates, with notable confidence intervals.6
The data presented is measured relative to concentrations in 1960 (where 1960 is equal to 0). ODS can have a significant lifetime in the atmosphere, for some between 50 and 100 years on average. This means that despite reductions in ODS emissions (and eventually complete phase-out of these substances), equivalent stratospheric chlorine (ESC) concentrations are expected to remain higher than 1960 levels through to the end of the century. However, it’s expected that they peaked in the early 2000s and will continue to slowly decline throughout this period.
In the chart, we also see stratospheric ozone concentrations relative to 1960 (1960 = 0). As a global average concentration, it’s expected that ozone levels will return to their 1960 levels around mid-century. Antarctica, where ozone depletion has been most severe due to very low temperatures is expected to recover much more slowly. It’s projected that Antarctic ozone concentrations will only begin to approach 1960 levels by the end of the century.
Have countries been misreporting emissions?
The story of international cooperation and action on addressing ozone depletion is a positive one: the Vienna Convention was the first Convention to receive universal ratification. Over the last few decades, we have seen a dramatic decline in emissions of ozone-depleting substances.
However, a 2018 study published in Nature reported “an unexpected and persistent increase in global emissions of ozone-depleting CFC-11”.7
Montzka et al. (2018) reported that since 2012 there had been an unexpected increase in emissions of trichlorofluoromethane (CFC-11), a historically dominant source of ozone-depleting substances, since 2012.
How was an increase in emissions detected?
Atmospheric concentrations of CFC-11 have been measured and traced back to the 1990s via air collection and analysis with automated onsite instrumentation, such as with gas chromatography coupled with electron capture detection (GC–ECD). This allows us to track atmospheric concentrations over time. Using statistics on reported emissions of CFC-11 submitted by parties to the Montreal Protocol, it is possible to construct estimates and projections of what change in atmospheric concentration should occur based on such levels of emissions. The World Meteorological Organization (WMO) publishes these estimates on multi-year timeframes.
In the chart, we see the annual change (in percent) of measured concentrations of CFC-11 (shown as the solid line). Also shown is the WMO 2014 projections/expectations of the rate of change in concentration based on reported emissions to the Montreal Protocol (shown as the dashed line). As we see, actual and expected concentration changes map closely over the period up to 2011. Since 2012, however, the annual rate of decline in concentrations has fallen (almost halved from -0.8 percent to -0.4 percent per year). This is highly inconsistent with the expected rate of change which would have resulted in the case that reported emissions to the Montreal Protocol were correct. This inconsistency between actual and expected rate of change (particularly in the case of a slowdown in concentration decline) suggests an increase in global emissions (despite reports close to zero since 2006).
Annual change in atmospheric CFC-11 concentrations
Annual change in atmospheric trichlorofluoromethane (CFC-11) concentrations, given as actual measured
concentration change (solid line), and expected/projected rate of change based on reported emissions of
parties to the Montreal Protocol (dashed line). Since 2012, concentrations have fallen at a lower rate than
expected, suggesting unreported sources of CFC-11 emissions.
199520182000200520102015-1.2%-1%-0.8%-0.6%-0.4%-0.2%0%WorldCC BY
Source: Montzka et al. 2018
19952018
- CHART
- TABLE
- SOURCES
- DOWNLOAD
Where are such emissions coming from?
It’s challenging to definitively attribute emissions sources to a particular geographical location for mixed global gases. However, some additional measurements allowed the authors to provide an informed estimate. Using combined CFC-11 measurements in the Northern and Southern Hemisphere and atmospheric transport models, the authors suggested the likely source of additional CFC-11 emissions was from the Northern Hemisphere. This was further supported by data from the Mauna Loa Observatory (MLO) in Hawaii, which also provides measurements of other chemical emissions. In correlating chemical pollution tracers and CFC-11 emissions, the authors suggest there is strong evidence that the source of increased CFC-11 emissions is Eastern Asia.
Montzka et al. (2018) suggest their evidence is inconsistent with the Montreal Protocol: “These considerations suggest that the increased CFC-11 emissions arise from new production not reported to UNEP’s Ozone Secretariat, which is inconsistent with the agreed phase-out of CFC production in the Montreal Protocol by 2010.”9
Will recent emissions hinder progress on ozone recovery?
How much of an impact will recent emissions of CFC-11 have on ozone layer recovery? The long-term impact of emissions for the ozone layer will depend on how long-continued emissions of CFC-11 persist.
In the chart, we show the absolute concentrations of CFC-11 (as opposed to the annual rate of change, shown above) in terms of actual measurements (solid lines, for both hemispheres) and projections (dashed line). Here you see that despite recent emissions, total concentrations continue to fall but at a notably slower rate than expected.
Montzka et al. (2018) note that this is expected to result in a delay to ozone recovery. However, this could be minimized (to the span of a few years) if emissions are now rapidly reduced (and return close to zero, as reported within the Ozone Secretariat).
Nonetheless, the capacity to identify where atmospheric concentrations and reported emissions are inconsistent is an important step in itself; it makes it clear that our measurement infrastructure does not allow misreporting to go unnoticed.
Why is ozone depletion more severe at high latitudes?
Although ozone depletion has been a global issue, there are significant differences in the distribution of ozone layer depletion across the world. Overall, ozone depletion increases with latitude with low levels of depletion at the equator and tropics, and the highest depletion at the poles. Although depletion has occurred over both the Antarctic and Arctic poles, Antarctica has experienced the most severe development of the ‘ozone hole’. Why is this the case?
An important condition for ozone depletion is very cold atmospheric temperatures. This factor alone explains the concentration of ozone depletion at the poles rather than at lower latitudes. Ozone depletion has been most severe over Antarctica because it provides the unique temperature and chemical conditions for effective ozone destruction by halogen gases.
Formation of the ‘ozone hole’ requires ‘polar stratospheric clouds’ (PSCs) to form; this occurs when temperatures fall below their formation temperature of around -78°C. This occurs for only 1-2 months in Arctic regions, but across 5 to 6 months in Antarctica through winter and early spring. The liquid and solid particles in PSCs allow highly reactive chlorine gas to be formed when halogen gases and sunlight are present. This highly reactive chlorine gas is then very effective in breaking down stratospheric ozone. It is these unique conditions through the winter and early spring that result in high ozone destruction over Antarctica.
Impacts on UV irradiation
The primary concern of the ‘ozone hole’ has been its impact on the quantity of UV-B radiation reaching Earth’s surface. As discussed earlier in this entry, stratospheric ozone plays a fundamental role in protecting surface lifeforms from exposure to harmful levels of UV-B radiation. This ‘good ozone’ typically absorbs 97-99 percent of incoming UV-B radiation.
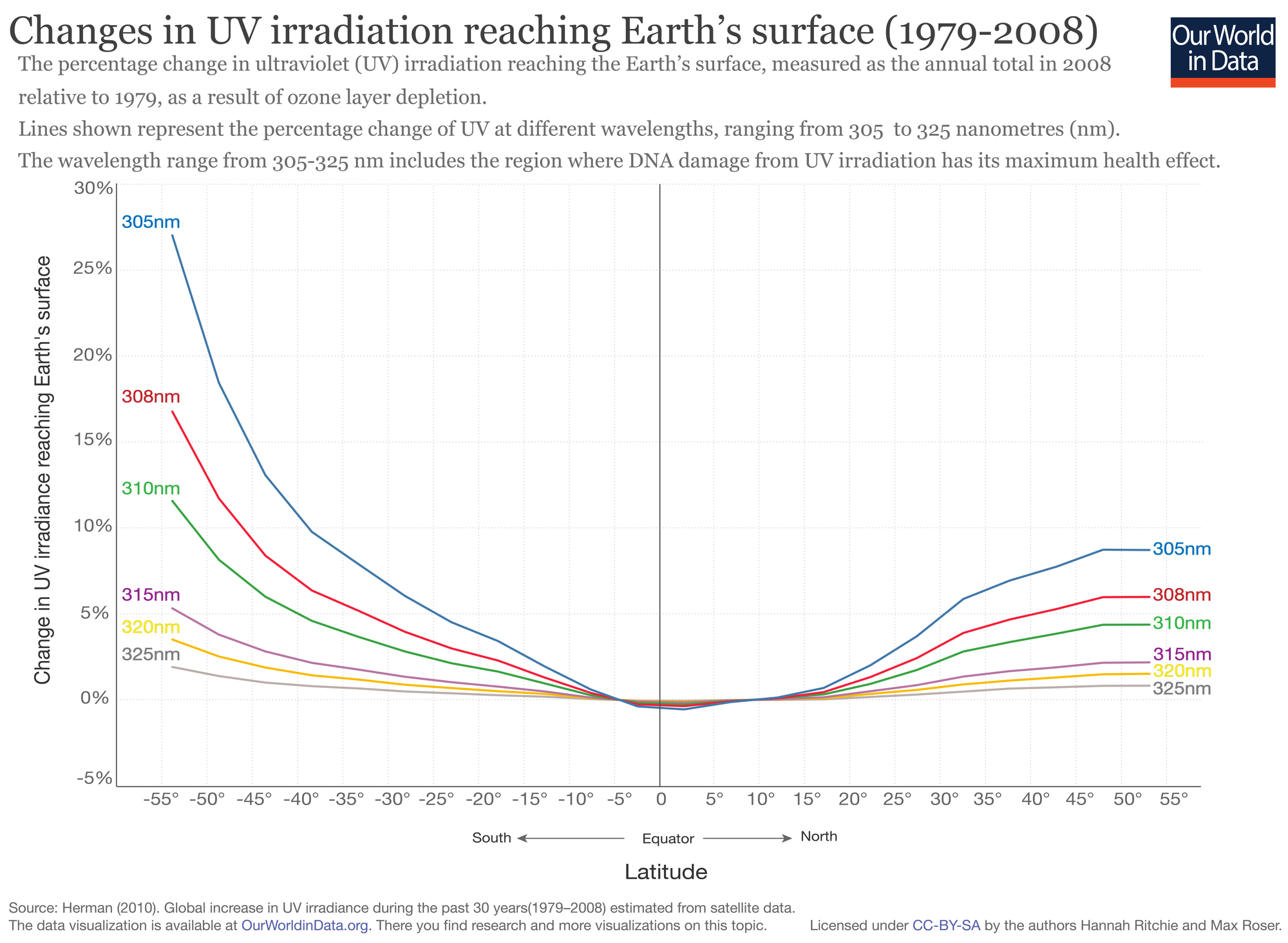
The primary concern of the ‘ozone hole’ has been its impact on the quantity of UV-B radiation reaching Earth’s surface. As discussed earlier in this entry, stratospheric ozone plays a fundamental role in protecting surface lifeforms from exposure to harmful levels of UV-B radiation. This ‘good ozone’ typically absorbs 97-99 percent of incoming UV-B radiation.
Depletion of stratospheric ozone has therefore had an impact on the quantity of UV irradiation reaching Earth’s surface. In the figure, we show the average percentage change in UV irradiation reach the surface in 2008 relative to levels in 1979.11
This is shown across latitudes (extending from -60° (South) to 60° (North), with each line representing the change for a given wavelength of UV irradiation. Shown are the changes across wavelengths from 305 to 325 nanometres, which is the region where DNA damage from UV irradiation has its largest health impacts.
Here we see that the largest increases in UV irradiation have been at high latitudes — particularly in the South Hemisphere. At 305 nm, for example, the irradiance at high Southern latitudes increased by more than 25 percent over this period. At the Northern poles, this was much less at between 5 to 10 percent.
Impacts on skin cancer risk
Ozone layer protection from UV-B irradiation is critical to the health of many lifeforms, including human health. Ozone depletion (and the subsequent increase in UV-B irradiation, as discussed above), can increase negative health impacts such as skin cancer, and other implications such as sunburn and skin ageing.
Using combined ozone, UV and dose-response models, numerous studies have attempted to quantify the potential increase in skin cancer cases as a result of ozone depletion. Such studies also attempt to quantify the number of skin cancer cases avoided as a result of international action through the Montreal Protocol to reduce ODS consumption.
Dijk et al. (2013) quantified the total number of cases of skin cancer avoided as a result of the Montreal Protocol and its subsequent amendments.
The study estimates that by 2030, two million cases worldwide will be avoided per year as a result of ODS reduction from the Montreal Protocol and its later revisions.
One of the first studies to attempt to quantify excess skin cancer cases, despite being published in the 1990s correlates well with results from recent studies.
In the chart, we present data on the estimated number of excess skin cancer cases per million people in the United States (estimates for Northern Europe can also be viewed using the “change region” button) with projections through to 2100.
This is shown for three scenarios: the assumption of no protocol/restrictions on ODS consumption; the initial Montreal Protocol treaty, and the later Copenhagen Amendment which increased ambition for ODS reduction.
Co-benefits on greenhouse gas emissions
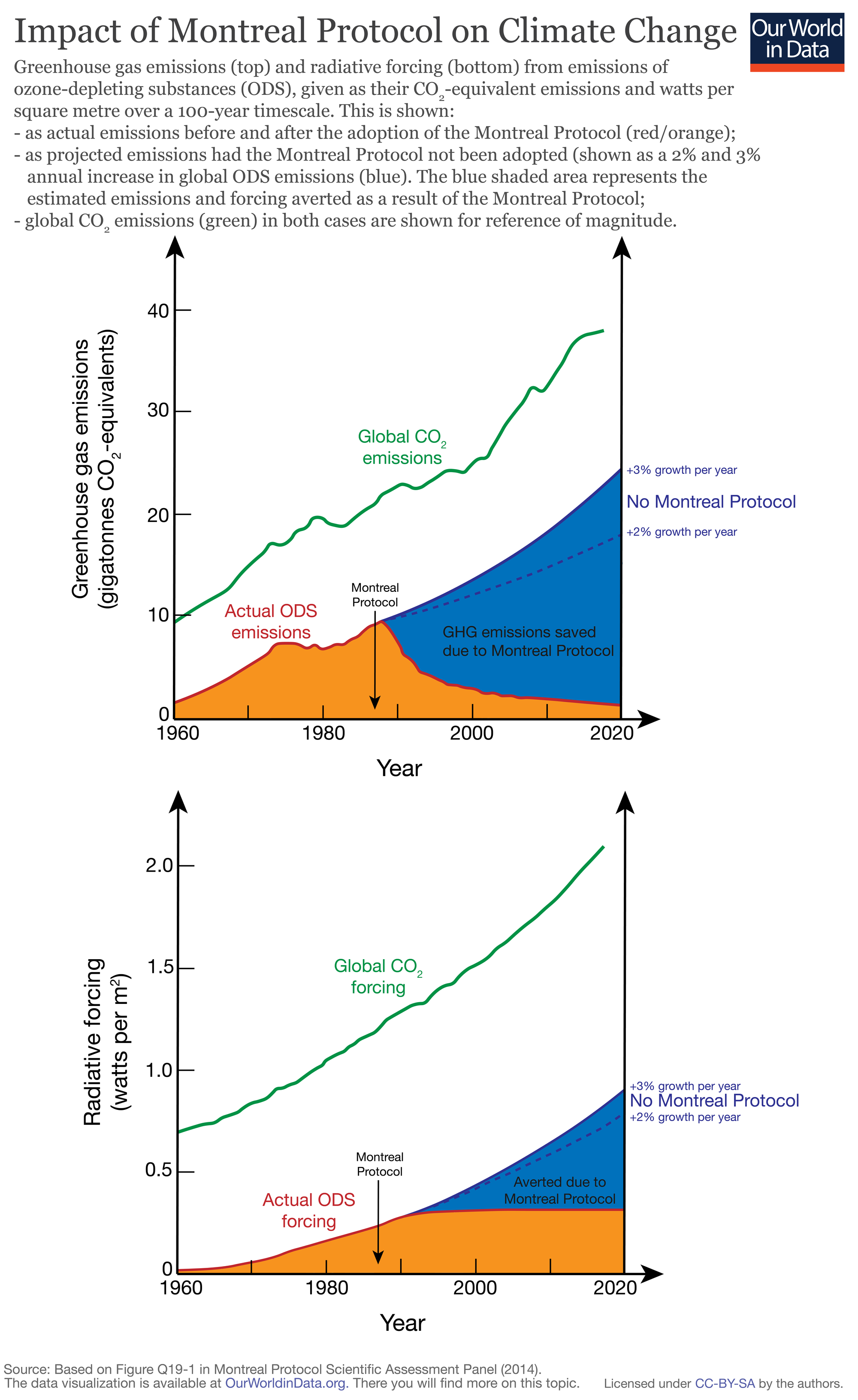
The primary role of the Vienna Convention, Montreal Protocol and its subsequent revisions was to protect the depletion of the stratospheric ozone layer. However, the ambition to reduce and eventually eliminate the use of ozone-depleting substances (ODS) has also produced co-benefits for greenhouse gas reduction.
Chlorofluorocarbons (CFCs) and hydrochlorofluorocarbons (HCFCs) and other halogen gases typically have a high global warming potential (GWP): they are powerful greenhouse gases with a high capacity to trap heat in the Earth’s atmosphere. With a GWP up to 10,000 times higher than carbon dioxide (CO2), these gases can have a notable impact on total greenhouse gas emissions, even in very small concentrations.
The reduction in ODS, in particular, CFCs has therefore had a significant impact on greenhouse gas reduction in recent years. In fact, several sources estimate that the climate benefits of the Montreal Protocol have been five to six times that of the Kyoto Protocol.
[The Kyoto Protocol was the international treaty for the United Nations Framework for Climate Change adopted in 1997, specifically designed to reduce greenhouse gas emissions].
In the chart, we visualize the impact of the reduction in ODS emissions for climate change in terms of greenhouse gas emissions (in carbon dioxide equivalents), and radiative forcing (measured in watts per square metre). This is based on the latest report from the World Meteorological Organisation (WMO) on the Scientific Assessment of Ozone Depletion.
In red/orange we show the actual trends in ozone-depleting substances, with the very obvious impact of the implementation of the Montreal Protocol on the reduction of GHG emissions and plateauing of radiative forcing. Blue trends map the estimated impact had the Montreal Protocol not been adopted: it is based on the assumption of a 2 to 3 percent (dashed and solid line) increase in annual production of halogen substances. The blue area, therefore, represents the estimated quantity of GHG or radiative forcing averted as a result of the Montreal Protocol.
The greenhouse gas emissions averted as a result of the reduction in ODSs is substantial: it’s estimated that without the Montreal Protocol, radiative forcing from ODSs could have reached 0.60 to 0.65 W/m2 in 2010 which was approximately 35 percent that of CO2 emissions.
GHG emissions from ODSs in 2010 could have reached approximately 10 billion tonnes of CO2-equivalents (which would have been five times the annual target of the Kyoto Protocol for the 2008-2012 period).
If increasing trends of ODSs had continued through to 2020, the WMO project that total GHG emissions from halogen gases could have approximated 20 billion tonnes of CO2-equivalents (relative to 36 billion tonnes of CO2 in 2017) — this would have been an increase of more than 50 percent to global GHG emissions.
Following the phaseout of CFCs, HFCs (which do not deplete ozone) have been increasingly substituted for HCFCs (which do deplete ozone) as part of the Montreal Protocol. This means HFC emissions have increased notably in recent years. However, HFCs have a large global-warming potential (as shown in the table in the Data Definitions section); and could begin to cancel out climate benefits made by the rapid reduction of CFCs and HCFCs in recent decades.
If we are to preserve the climate benefits of the Montreal Protocol, further emissions and potential substitutes for CFC products will be important in the coming years.
Definitions & Measurement
Units of ozone-depleting substance emissions
Ozone-depleting substances (ODS) are typically measured in ‘ozone depletion potential' (ODP) tonnes. ODP tonnes attempt to standardize and correct for the differences in the potential ozone destruction across different substances.
In the table in the section below, we provide a list of substances and their respective ODP values; ODP values provide a measure of the relative destruction potential of one tonne of that substance relative to chlorofluorocarbon-11 (CFC-11). CFC-11 is therefore given a value of 1. For example, HCFCs have an ODP ranging from 0.01 to 0.1, meaning that one tonne of HCFC gas causes 10 to 100 times less ozone destruction than one tonne of CFC-11.
To calculate ODS tonnes, emissions of a given substance in tonnes is multiplied by its ODP value. For example, ten tonnes of Halon-1301 would result in 152 ODP tonnes (= 10 * 15.2). The sum of all the halogen gases in ODP tonnes provides a total measure of ozone-depleting substance emissions.
ODP tonnes can also be reported as CFC-11-equivalents since all values are normalized to the depletion potential of CFC-11 (which is given an ODP value of 1).
Emissions vs. production vs. concentration
In the entry above we present data on production/consumption, emissions, and stratospheric concentrations of ozone-depleting substances. It’s important to note the distinction between these measures, and why they are not equivalent.
Production/consumption of ozone-depleting substances refers to the primary production of new materials or products containing any of the halogen gases noted as ODS. The important aspect to note here is that this relates to the production or release of new/recent substances.
Emissions of ozone-depleting substances can be natural or man-made. Small, relatively consistent levels of ozone-depleting substances are emitted through natural processes. Most discussion in relation to ODS emissions, therefore, focuses on man-made emissions which can be controlled.
Emissions of ODS result from the production/consumption of new materials or products, but they can also occur in the absence of new production. The use of halogen gases in products such as refrigerants, solvents and foams has been persistent since the early 20th century. These gases still reside in some products which are liable to leakage over time. In the case of chlorofluorocarbons, these are called ‘CFC banks’.
The storage and persistence of ‘old’ reservoirs/sources of ozone-depleting substances mean that emissions can continue over time, even in the absence of new production of these substances.
Concentrations of ozone-depleting substances in the atmosphere are determined by the stock of substances, in addition to the balance of inputs and outputs over time. The lifetime of gases in the atmosphere plays an important role in understanding how their concentrations change over time.
Even once emissions of ODS cease; why does the concentration of these substances not return to zero? In the table, in the following section, we detail the average atmospheric ‘lifetime’ of specific substances. For many substances, this timeframe is on the scale of decades (up to a century). As a result, even if emissions were halted, depleting substances (which were previously emitted) would continue to persist in the stratosphere. It will therefore take many decades for full recovery as substances are removed.
Ozone-depleting substances
Gas | Ozone depleting potential (ODP) | Global warming potential (GWP100) | Atmospheric lifetime (years) |
---|---|---|---|
Chlorine gases | |||
CFC-11 | 1 | 5160 | 52 |
CFC-12 | 0.73 | 10300 | 102 |
CFC-113 | 0.81 | 6080 | 93 |
Carbon tetrachloride (CCl4) | 0.72 | 1730 | 26 |
HCFCs | 0.01-0.1 | 800-2070 | 1-18 |
Methyl chloroform (CH3CCl3) | 0.14 | 153 | 5 |
Methyl chloride (CH3Cl) | 0.015 | 11 | 0.9 |
Very short-lived Cl-containing gases | Very low | <1 | <0.5 |
Bromine gases | |||
Halon-1301 | 15.2 | 1.4-2 | 72 |
Halon-1211 | 6.9 | 0.3-9.3 | 16 |
Methyl bromide (CH3Br) | 0.57 | 85 | 0.8 |
Very short-lived Br-containing gases (e.g. CHBr3) | Very low | 260-1080 | <0.5 |
Hydrofluorocarbons (HFCs) | |||
HFC-134a | 0 | 1360 | 14 |
HFC-23 | 0 | 12500 | 228 |
HFC-143a | 0 | 5080 | 51 |
HFC-125 | 0 | 3450 | 31 |
HFC-152a | 0 | 148 | 1.6 |
HFC-32 | 0 | 700 | 5.4 |
In the table, we list the range of ozone-depleting substances (ODS) with their corresponding ozone-depleting potential (ODP) per tonne (measured relative to CFC-11 which is equal to 1); their global warming potential (GWP) and atmospheric lifetime (in years).
Data sources
United Nations Environment Programme (UNEP)
- Data: Annual emissions of ozone-depleting substances
- Geographical coverage: Global- by country
- Timespan: 1989-2014
- Available at: http://ede.grid.unep.ch/
NASA Ozone Watch
- Data: Concentration of atmospheric ozone and ozone hole area
- Geographical coverage: Global
- Timespan: 1979-2017
- Available at: NASA Ozone Hole Watch
NASA Monthly Ozone Maps
- Data: Monthly satellite maps of the Antarctic ozone
- Geographical coverage: Antarctic pole
- Time span: 1979-present (monthly)
- Available at: NASA Ozone Hole Watch Satellite Imaging
Ozone Secretariat Scientific Assessment Panel
- Data: Synthesis reports on ‘Twenty Questions and Answers About the Ozone Layer’
- Time span: Published every four years (2006; 2010; 2014; 2018)
- Available at: Ozone Secretariat Scientific Assessment Panel